According to the known laws of physics, the universe we see today should be dark, empty and quiet. There should be no stars, no planets, no galaxies and no life—just energy and simple particles diffusing further and further into an expanding universe.
And yet, here we are.
Cosmologists calculate that roughly 13.8 billion years ago, our universe was a hunk of thick, hot energy with no boundaries and its own rules. But then, in less than a microsecond, it matured, and the fundamental laws and properties of matter arose from the pandemonium. How did our elegant and intricate universe emerge?
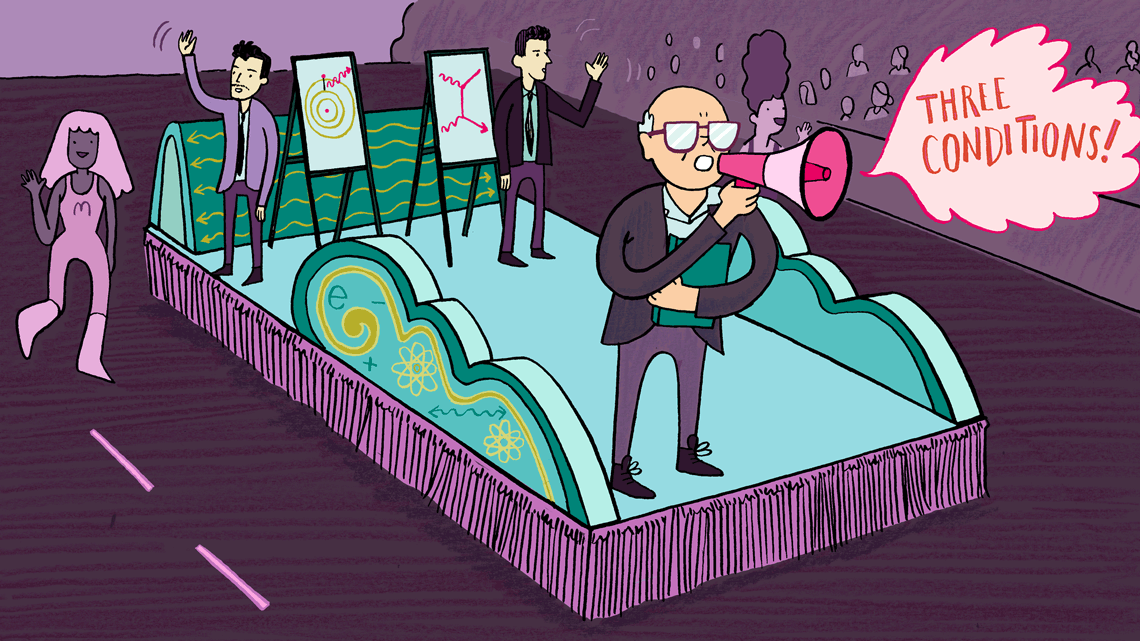
The three conditions
The question “How is it here?” alludes to a conundrum that arose during the development of quantum mechanics.
In 1928 Paul Dirac combined quantum theory and special relativity to predict the energy of an electron moving near the speed of light. But his equations produced two equally favorable answers: one positive and one negative. Because energy itself cannot be negative, Dirac mused that perhaps the two answers represented the particle’s two possible electric charges. The idea of oppositely charged matter-antimatter pairs was born.
Meanwhile, about six minutes away from Dirac’s office in Cambridge, physicist Patrick Blackett was studying the patterns etched in cloud chambers by cosmic rays. In 1933 he detected 14 tracks that showed a single particle of light colliding with an air molecule and bursting into two new particles. The spiral tracks of these new particles were mirror images of each other, indicating that they were oppositely charged. This was one of the first observations of what Dirac had predicted five years earlier—the birth of an electron-positron pair.
Today it’s well known that matter and antimatter are the ultimate wonder twins. They’re spontaneously born from raw energy as a team of two and vanish in a silent poof of energy when they merge and annihilate. This appearing-disappearing act spawned one of the most fundamental mysteries in the universe: What is engraved in the laws of nature that saved us from the broth of appearing and annihilating particles of matter and antimatter?
“We know this cosmic asymmetry must exist because here we are,” says Jessie Shelton, a theorist at the University of Illinois. “It’s a puzzling imbalance because theory requires three conditions—which all have to be true at once—to create this cosmic preference for matter.”
In the 1960s physicist Andrei Sakharov proposed this set of three conditions that could explain the appearance of our matter-dominated universe. Scientists continue to look for evidence of these conditions today.
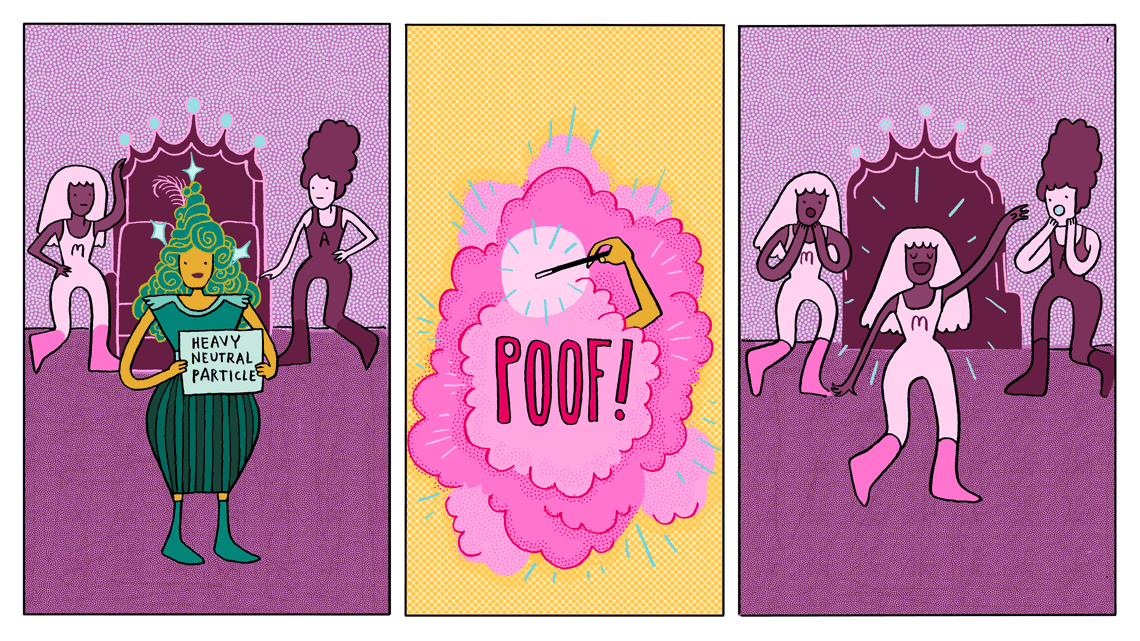
1. Breaking the tether
The first problem is that matter and antimatter always seem to be born together. Just as Blackett observed in the cloud chambers, uncharged energy transforms into evenly balanced matter-antimatter pairs. Charge is always conserved through any transition. For there to be an imbalance in the amounts of matter and antimatter, there needs to be a process that creates more of one than the other.
“Sakharov’s first criterion essentially says that there must be some new process that converts antimatter into matter, or vice versa,” says Andrew Long, a postdoctoral researcher in cosmology at the University of Chicago. “This is one of the things experimentalists are looking for in the lab.”
In the 1980s, scientists searched for evidence of Sakharov’s first condition by looking for signs of a proton decaying into a positron and two photons. They have yet to find evidence of this modern alchemy, but they continue to search.
“We think that the early universe could have contained a heavy neutral particle that sometimes decayed into matter and sometimes decayed into antimatter, but not necessarily into both at the same time,” Long says.
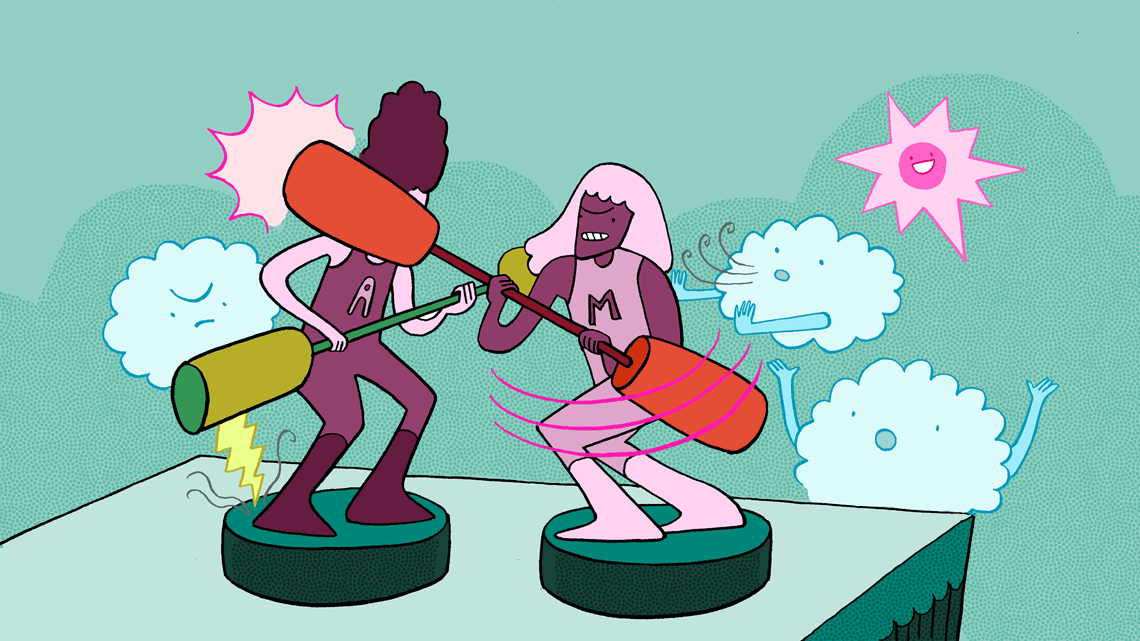
2. Picking a favorite
Matter and antimatter cannot co-habitate; they always annihilate when they come into contact. But the creation of just a little more matter than antimatter after the Big Bang—about one part in 10 billion—would leave behind the ingredients needed to build the entire visible universe.
How could this come about? Sakharov’s second criterion dictates that the matter-only process outlined in his first criterion must be more efficient than the opposing antimatter process. And specifically, “we need to see a favoritism for the right kinds of matter to agree with astronomical observations,” Shelton says.
Observations of light left over from the early universe and measurements of the first lightweight elements produced after the Big Bang show that the discrepancy must exist in a class of particles called baryons: protons, antiprotons and other particles constructed from quarks.
“These are snapshots of the early universe,” Shelton says. “From these snapshots, we can derive the density and temperature of the early universe and calculate the slight difference between the number of baryons and antibaryons.”
But this slight difference presents a problem. While there are some tiny discrepancies between the behavior of particles and their antiparticle counterparts, these idiosyncrasies are still consistent with the Standard Model and are not enough to explain the origin of the cosmic imbalance nor the universe’s tenderness towards matter.
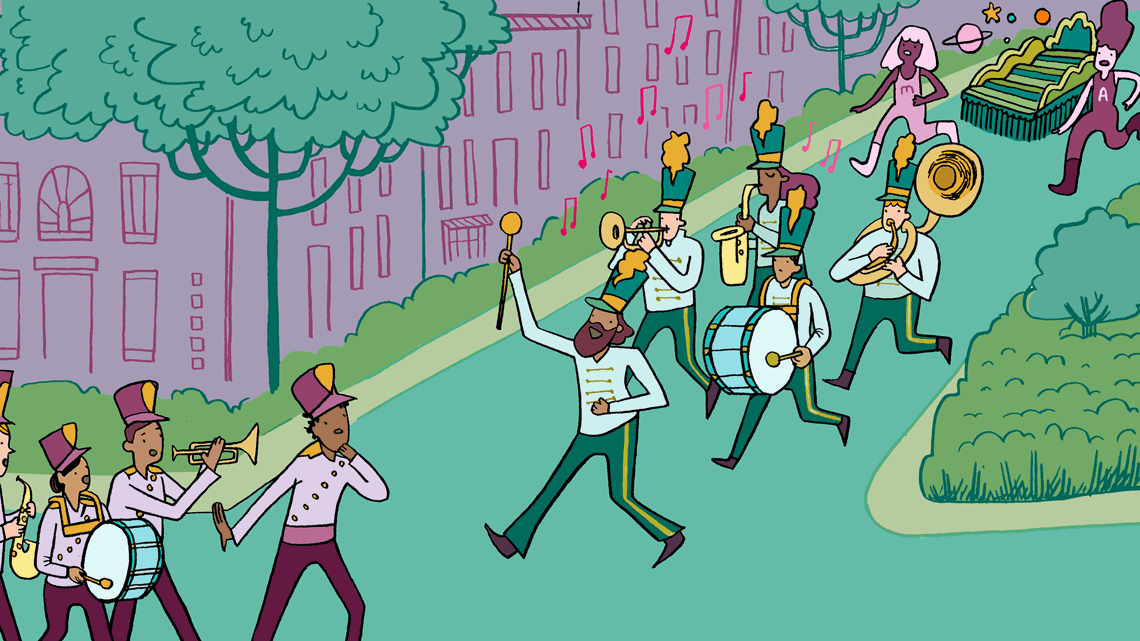
3. Taking a one-way street
In particle physics, any process that runs forward can just as easily run in reverse. A pair of photons can merge and morph into a particle and antiparticle pair. And just as easily, the particle and antiparticle pair can recombine into a pair of photons. This process happens all around us, continually. But because it is cyclical, there is no net gain or loss for a type of matter.
If this were always true, our young universe could have been locked in an infinite loop of creation and destruction. Without something slamming the brakes on these cycles at least for a moment, matter could not have evolved into the complex structures we see today.
“For every stitch that’s knit, there a simultaneous tug on the thread,” Long says. “We need a way to force the reaction to move forward and not simultaneously run in reverse at the same rate.”
Many cosmologists suspect that the gradual expansion and cooling of the universe was enough to lock matter into being, like a supersaturated sweet tea whose sugar crystals drop to the bottom of the glass as it cools (or in the “freezing” interpretation, like a sweet tea that instantly freezes into ice, locking sugar crystals in place without giving them a chance to dissolve).
Other cosmologists think that the plasma of the early universe may have contained bubbles that helped separate matter and antimatter (and then served as incubators for particles to acquire mass).
Several experiments at CERN are looking for evidence that the universe meets Sakharov’s three conditions. For instance, several precision experiments at CERN’s Antimatter Factory are looking for minuscule differences between the intrinsic characteristics of protons and antiprotons. The LHCb experiment at the Large Hadron Collider is examining the decay patterns of unstable matter and antimatter particles.
Shelton and Long both hope that more research from experiments at the LHC will be the key to building a more complete picture of our early universe.
LHC experiments could discover that the Higgs field served as the lock that halted the early universe’s perpetually evolving and devolving particle soup—especially if the field contained bubbles that froze faster than others, providing cosmic petri dishes in which matter and antimatter could evolve differently, Long says. “More measurements of the Higgs boson and the fundamental properties of matter and antimatter will help us develop better theories and a better understanding of what and where we come from.”
What exactly transpired during the birth of our universe may always remain a bit of an enigma, but we continue to seek new pieces of this formidable puzzle.